Thermoelectric generator
Thermoelectric effect |
---|
![]() |
Principles
|
A thermoelectric generator (TEG), also called a Seebeck generator, is a solid state device that converts heat (temperature differences) directly into electrical energy through a phenomenon called the Seebeck effect (a form of thermoelectric effect). Thermoelectric generators function like heat engines, but are less bulky and have no moving parts. However, TEGs are typically more expensive and less efficient.[1]
Thermoelectric generators could be used in power plants in order to convert waste heat into additional electrical power and in automobiles as automotive thermoelectric generators (ATGs) to increase fuel efficiency. Another application is radioisotope thermoelectric generators which are used in space probes, which has the same mechanism but use radioisotopes to generate the required heat difference.[1]
History
In 1821, Thomas Johann Seebeck discovered that a thermal gradient formed between two dissimilar conductors can produce electricity.[2] At the heart of the thermoelectric effect is the fact that a temperature gradient in a conducting material results in heat flow; this results in the diffusion of charge carriers. The flow of charge carriers between the hot and cold regions in turn creates a voltage difference. In 1834, Jean Charles Athanase Peltier discovered the reverse effect, that running an electric current through the junction of two dissimilar conductors could, depending on the direction of the current, cause it to act as a heater or cooler.[3]
Construction
Thermoelectric power generators consist of three major components: thermoelectric materials, thermoelectric modules and thermoelectric systems that interface with the heat source.[4]
Thermoelectric materials
Thermoelectric materials generate power directly from heat by converting temperature differences into electric voltage. These materials must have both high electrical conductivity (σ) and low thermal conductivity (κ) to be good thermoelectric materials. Having low thermal conductivity ensures that when one side is made hot, the other side stays cold, which helps to generate a large voltage while in a temperature gradient. The measure of the magnitude of electrons flow in response to a temperature difference across that material is given by the Seebeck coefficient (S). The efficiency of a given material to produce a thermoelectric power is governed by its “figure of merit” zT = S2σT/κ.
For many years, the main three semiconductors known to have both low thermal conductivity and high power factor were bismuth telluride (Bi2Te3), lead telluride (PbTe), and silicon germanium (SiGe). These materials have very rare elements which make them very expensive compounds.
Today, the thermal conductivity of semiconductors can be lowered without affecting their high electrical properties using nanotechnology. This can be achieved by creating nanoscale features such as particles, wires or interfaces in bulk semiconductor materials. However, the manufacturing processes of nano-materials is still challenging.
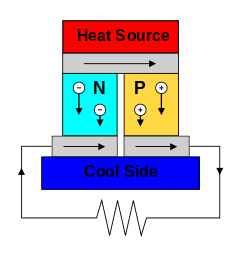
Thermoelectric module
A thermoelectric module is a circuit containing thermoelectric materials that generate electricity from heat directly. A thermoelectric module consists of two dissimilar thermoelectric materials joining in their ends: an n-type (negatively charged); and a p-type (positively charged) semiconductors. A direct electric current will flow in the circuit when there is a temperature difference between the two materials. Generally, the current magnitude has a proportional relationship with the temperature difference. (i.e., the more the temperature difference, the higher the current.)
In application, thermoelectric modules in power generation work in very tough mechanical and thermal conditions. Because they operate in very high temperature gradient, the modules are subject to large thermally induced stresses and strains for long periods of time. They also are subject to mechanical fatigue caused by large number of thermal cycles.
Thus, the junctions and materials must be selected so that they survive these tough mechanical and thermal conditions. Also, the module must be designed such that the two thermoelectric materials are thermally in parallel, but electrically in series. The efficiency of thermoelectric modules are greatly affected by its geometrical design.
Thermoelectric system
Using thermoelectric modules, a thermoelectric system generates power by taking in heat from a source such as a hot exhaust flue. In order to do that, the system needs a large temperature gradient, which is not easy in real-world applications. The cold side must be cooled by air or water. Heat exchangers are used on both sides of the modules to supply this heating and cooling.
There are many challenges in designing a reliable TEG system that operates at high temperatures. Achieving high efficiency in the system requires extensive engineering design in order to balance between the heat flow through the modules and maximizing the temperature gradient across them. To do this, designing heat exchanger technologies in the system is one of the most important aspects of TEG engineering. In addition, the system requires to minimize the thermal losses due to the interfaces between materials at several places. Another challenging constraint is avoiding large pressure drops between the heating and cooling sources.
After the DC power from the TE modules passes through an inverter, the TEG produces AC power, which in turn, requires an integrated power electronics system to deliver it to the customer.
Materials for TEG
Only a few known materials to date are identified as thermoelectric materials. Most thermoelectric materials today have a ZT, the figure of merit, value of around unity, such as in Bismuth Telluride (Bi2Te3) at room temperature and lead telluride (PbTe) at 500-700K. However, in order to be competitive with other power generation systems, TEG materials should have zT of 2-3 range. Most research in thermoelectric materials has focused on increasing the Seebeck coefficient (S) and reducing the thermal conductivity, especially by manipulating the nanostructure of the thermoelectric materials. Because the thermal and electrical conductivity correlate with the charge carriers, new means must be introduced in order to conciliate the contradiction between high electrical conductivity and low thermal conductivity as indicated.[5]
When selecting materials for thermoelectric generation, a number of other factors need to be considered. During operation, ideally the thermoelectric generator has a large temperature gradient across it. Thermal expansion will then introduce stress in the device which may cause fracture of the thermoelectric legs, or separation from the coupling material. The mechanical properties of the materials must be considered and the coefficient of thermal expansion of the n and p-type material must be matched reasonably well. In segmented thermoelectric generators, the material's compatibility must also be considered. A material's compatibility factor is defined as
.[6] When the compatibility factor from one segment to the next differs by more than a factor of about two, the device will not operate efficiently. The material parameters determining s (as well as zT) are temperature dependent, so the compatibility factor may change from the hot side to the cold side of the device, even in one segment. This behavior is referred to as self-compatibility and may become important in devices design for low temperature operation.
In general, thermoelectric materials can be categorized into conventional and new materials:
Conventional materials
There are many TEG materials that are employed in commercial applications today. These materials can be divided into three groups based on the temperature range of operation:
- Low temperature materials (up to around 450K): Alloys based on Bismuth (Bi) in combinations with Antimony (Sb), Tellurium (Te) or Selenium (Se).
- Intermediate temperature (up to 850K): such as materials based on alloys of Lead (Pb)
- Highest temperatures material (up to 1300K): materials fabricated from silicon germanium (SiGe) alloys.
Although these materials still remain the cornerstone for commercial and practical applications in thermoelectric power generation, significant advances have been made in synthesizing new materials and fabricating material structures with improved thermoelectric performance. Recent research have focused on improving the material’s figure-of-merit (zT), and hence the conversion efficiency, by reducing the lattice thermal conductivity.[5]
New materials
Researchers are trying to develop new thermoelectric materials for power generation by improving the figure-of-merit zT. One example of these materials is the semiconductor compound ß-Zn4Sb3, which possesses an exceptionally low thermal conductivity and exhibits a maximum zT of 1.3 at a temperature of 670K. This material is also relatively inexpensive and stable up to this temperature in a vacuum, and can be a good alternative in the temperature range between materials based on Bi2Te3 and PbTe.[5]
Beside improving the figure-of-merit, there is increasing focus to develop new materials by increasing the electrical power output, decreasing cost and developing environmentally friendly materials. For example, when the fuel cost is low or almost free, such as in waste heat recovery, then the cost per watt is only determined by the power per unit area and the operating period. As a result, it has initiated a search for materials with high power output rather than conversion efficiency. For example, the rare earth compounds YbAl3 has a low figure-of-merit, but it has a power output of at least double that of any other material, and can operate over the temperature range of a waste heat source.[5]
Novel Processing
In order to increase the figure of merit (zT), a material’s thermal conductivity should be minimized while its electrical conductivity and Seebeck coefficient is maximized. In most cases, methods to increase or decrease one property result in the same effect on other properties due to their interdependence.[7] A novel processing technique exploits the scattering of different phonon frequencies to selectively reduce lattice thermal conductivity without the typical negative effects on electrical conductivity from the simultaneous increased scattering of electrons. In a bismuth antimony tellurium ternary system, liquid-phase sintering is used to produce low-energy semicoherent grain boundaries, which do not have a significant scattering effect on electrons.[8] The breakthrough is then applying a pressure to the liquid in the sintering process, which creates a transient flow of the Te rich liquid and facilitates the formation of dislocations that greatly reduce the lattice conductivity.[8] The ability to selectively decrease the lattice conductivity results in reported zT values of 1.86 ± .15 which are a significant improvement over current commercial thermoelectric generators which have typical figures of merit closer to .3-.6.[9] These improvements highlight the fact that in addition to development of novel materials for thermoelectric applications, using different processing techniques to design microstructure is a viable and worthwhile effort. In fact, it often makes sense to work to optimize both composition and microstructure.[10]
Efficiency
The typical efficiency of TEGs is around 5–8%. Older devices used bimetallic junctions and were bulky. More recent devices use highly doped semiconductors made from bismuth telluride (Bi2Te3), lead telluride (PbTe),[11] calcium manganese oxide (Ca2Mn3O8),[12][13] or combinations thereof,[14] depending on temperature. These are solid-state devices and unlike dynamos have no moving parts, with the occasional exception of a fan or pump. For a discussion of the factors determining and limiting efficiency, and ongoing efforts to improve the efficiency, see the article Thermoelectric materials - Device efficiency.
Uses
Thermoelectric generators have a variety of applications. Frequently, thermoelectric generators are used for low power remote applications or where bulkier but more efficient heat engines such as Stirling engines would not be possible. Unlike heat engines, the solid state electrical components typically used to perform thermal to electric energy conversion have no moving parts. The thermal to electric energy conversion can be performed using components that require no maintenance, have inherently high reliability, and can be used to construct generators with long service free lifetimes. This makes thermoelectric generators well suited for equipment with low to modest power needs in remote uninhabited or inaccessible locations such as mountaintops, the vacuum of space, or the deep ocean.
- Common application is the use of thermoelectric generators on gas pipelines. For example, for cathodic protection, radio communication, and other telemetry. On gas pipelines for power consumption of up to 5 kW thermal generators are preferable to other power sources. The manufacturers of generators for gas pipelines are Gentherm Global Power Technologies (Formerly Global Thermoelectric), (Calgary, Canada) and TELGEN (Russia).
- Thermoelectric Generators are primarily used as remote and off-grid power generators for unmanned sites. They are the most reliable power generator in such situations as they do not have moving parts (thus virtually maintenance free), work day and night, perform under all weather conditions, and can work without battery backup. Although Solar Photovoltaic systems are also implemented in remote sites, Solar PV may not be a suitable solution where solar radiation is low, i.e. areas at higher latitudes with snow or no sunshine, areas with lots of cloud or tree canopy cover, dusty deserts, forests, etc.
- Gentherm Global Power Technologies (GPT) formerly known as Global Thermoelectric (Canada) has Hybrid Solar-TEG solutions where the Thermoelectric Generator backs up the Solar-PV, such that if the Solar panel is down and the backup battery backup goes into deep discharge then a sensor starts the TEG as a backup power source until the Solar is up again. The TEG heat can be produced by a low pressure flame fueled by Propane or Natural Gas.
- Many space probes, including the Mars Curiosity rover, generate electricity using a radioisotope thermoelectric generator whose heat source is a radioactive element.
- Cars and other automobiles produce waste heat (in the exhaust and in the cooling agents). Harvesting that heat energy, using a thermoelectric generator, can increase the fuel efficiency of the car. For more details, see the article: Automotive Thermoelectric Generators.
- In addition to automobiles, waste heat is also generated in many other places, such as in industrial processes and in heating (wood stoves, outdoor boilers, cooking, oil and gas fields, pipelines, and remote communication towers).
- Microprocessors generate waste heat. Researchers have considered whether some of that energy could be recycled.[15] (However, see below for problems that can arise.)
- Solar cells use only the high frequency part of the radiation, while the low frequency heat energy is wasted. Several patents about the use of thermoelectric devices in tandem with solar cells have been filed.[16] The idea is to increase the efficiency of the combined solar/thermoelectric system to convert the solar radiation into useful electricity.
- The Maritime Applied Physics Corporation in Baltimore, Maryland [17] is developing a thermoelectric generator to produce electric power on the deep-ocean offshore seabed using the temperature difference between cold seawater and hot fluids released by hydrothermal vents, hot seeps, or from drilled geothermal wells. A high reliability source of seafloor electric power is needed for ocean observatories and sensors used in the geological, environmental, and ocean sciences, by seafloor mineral and energy resource developers, and by the military.
- Ann Makosinski from British Columbia, Canada has developed several devices using Peltier tiles to harvest heat (from a human hand,[18] the forehead, and hot beverage[19]) that claims to generate enough electricity to power an LED light or charge a mobile device, although the inventor admits that the brightness of the LED light is not competitive with those on the market.[20]
Practical limitations
Besides low efficiency and relatively high cost, practical problems exist in using thermoelectric devices in certain types of applications resulting from a relatively high electrical output resistance, which increases self-heating, and a relatively low thermal conductivity, which makes them unsuitable for applications where heat removal is critical, as with heat removal from an electrical device such as microprocessors.
- High generator output resistance: In order to get voltage output levels in the range required by digital electrical devices, a common approach is to place many thermoelectric elements in series within a generator module. The element's voltages add, but so do their individual output resistance. The maximum power transfer theorem dictates that maximum power is delivered to a load when the source and load resistances are identically matched. For low impedance loads near zero ohms, as the generator resistance rises the power delivered to the load decreases. To lower the output resistance, some commercial devices place more individual elements in parallel and fewer in series and employ a boost regulator to raise the voltage to the voltage needed by the load.
- Low thermal conductivity: Because a very low thermal conductivity is required to transport thermal energy away from a heat source such as a digital microprocessor. The relatively high thermal conductivity of a generator module relative to copper and aluminum thermal conductors used in heat sinks means the thermoelectric generator impedes the waste heat removal causing the silicon device temperature to rise significantly.
- Cold-side heat removal with air: In air-cooled thermoelectric applications, such as when harvesting thermal energy from a motor vehicle's crankcase, the large amount of thermal energy that must be dissipated into ambient air presents a significant challenge. As a thermoelectric generator's cool side temperature rises, the device's differential working temperature decreases. Ast the temperature rises, the device's electrical resistance increases causing greater parasitic generator self-heating. In motor vehicle applications a supplementary radiator is sometimes used for improved heat removal, though the use of an electric water pump to circulate a coolant adds an additional parasitic loss to total generator output power. Water cooling the thermoelectric generator's cold side, as when generating thermoelectric power from the hot crank case of an inboard boat motor, would not suffer from this disadvantage. Water is a fare easier coolant to use effectively in contrast to air.
Future Market
While TEG technology has been used in military and aerospace applications for decades, new TE materials and systems are being developed to generate power using low or high temperatures waste heat, and that could provide a significant opportunity in the near future . These systems can also be scalable to any size and have lower operation and maintenance cost.
In general, investing in TEG technology is increasing rapidly. The global market for thermoelectric generators is estimated to be US$320 million in 2015. A recent study estimated that TEG is expected to reach $720 million in 2021 with a growth rate of 14.5%. Today, North America capture 66% of the market share and it will continue to be the biggest market in the near future.[21] However, Asia-Pacific and European countries are projected to grow at relatively higher rates. A study found that the Asia-Pacific market would grow at a Compound Annual Growth Rate (CAGR) of 18.3% in the period from 2015 to 2020 due to the high demand of thermoelectric generators by the automotive industries to increase overall fuel efficiency, as well as the growing industrialization in the region.[22]
Low power TEG or "Sub-watt" (i.e. generating up to 1 Watt peak) market is a growing part of the TEG market, capitalizing on latest technologies. Main applications are sensors, low power applications and more globally Internet of things applications. A specialized market research company indicated that 100 000 units have been shipped in 2014 and expects 9 million units per year by 2020.[23]
See also
References
- 1 2 Adroja, Mr Nikunj; B.Mehta, Prof Shruti; Shah, Mr Pratik (2015-03-01). "Review of thermoelectricity to improve energy quality". 2 - Issue 3 (March-2015). JETIR.
- ↑ See:
- Seebeck, T. J. (1825). "Magnetische Polarisation der Metalle und Erze durch Temperatur-Differenz (Magnetic polarization of metals and minerals by temperature differences)". Abhandlungen der Königlichen Akademie der Wissenschaften zu Berlin (Treatises of the Royal Academy of Sciences in Berlin). pp. 265–373.
- Seebeck, T. J. (1826). "Ueber die Magnetische Polarisation der Metalle und Erze durch Temperatur-Differenz," (On the magnetic polarization of metals and minerals by temperature differences),". Annalen der Physik und Chemie. 6: 1–20, 133–160, 253–286.
- ↑ Peltier (1834). "Nouvelles expériences sur la caloricité des courants électrique (New experiments on the heat effects of electric currents)". Annales de Chimie et de Physique. 56: 371–386.
- ↑ "How Thermoelectric Generators Work - Alphabet Energy". Alphabet Energy. Retrieved 2015-10-28.
- 1 2 3 4 Ismail, Basel I.; Ahmed, Wael H. (2009-01-01). "Thermoelectric Power Generation Using Waste-Heat Energy as an Alternative Green Technology". Recent Patents on Electrical & Electronic Engineering (Formerly Recent Patents on Electrical Engineering). 2 (1): 27–39. doi:10.2174/1874476110902010027.
- ↑ Snyder, G. (Oct 2003). "Thermoelectric Efficiency and Compatibility". Physical Review Letters. 91 (14). doi:10.1103/physrevlett.91.148301.
- ↑ Chatterji, Tapan. "Increasing the Thermoelectric Figure of Merit". ResearchGate. Retrieved 8 November 2016.
- 1 2 Kim, Sang (April 3, 2015). "Dense dislocation arrays embedded in grain boundaries for high-performance bulk thermoelectrics". Science. 348 (6230): 109–114. doi:10.1126/science.aaa4166. Retrieved 8 November 2016.
- ↑ Kim, D.S. (January 2008). "Solar refrigeration options – a state-of-the-art review". International Journal of Refrigeration. 31 (1): 3–15. Retrieved 8 November 2016.
- ↑ Cojocaru-Mirédin, Oana. "Thermoelectric Materials Design by controlling the microstructure and composition". Max-Planck Institut. Retrieved 8 November 2016.
- ↑ High-performance bulk thermoelectrics with all-scale hierarchical architectures
- ↑ "calcium manganese oxide Ca2Mn3O8". International Union of Crystallography. Retrieved 11 August 2015.
- ↑ "EspressoMilkCooler.com – TEG CMO 800°C & Cascade 600°C Hot Side Thermoelectric Power Modules". espressomilkcooler.com.
- ↑ High Temp Teg Power Modules Archived December 17, 2012, at the Wayback Machine.
- ↑ "Harvesting Wasted Heat in a Microprocessor Using Thermoelectric Generators: Modeling, Analysis and Measurement". 2008 Design, Automation and Test in Europe. doi:10.1109/DATE.2008.4484669.
- ↑ Kraemer, D; Hu, L; Muto, A; Chen, X; Chen, G; Chiesa, M (2008), "Photovoltaic-thermoelectric hybrid systems: A general optimization methodology", Applied Physics Letters, 92: 243503, doi:10.1063/1.2947591
- ↑ "MAPC". MAPC.
- ↑ "GSF 2013 : Project : The Hollow Flashlight". Google Science Fair. Retrieved 2015-12-25.
- ↑ "Thene-Drink: Capturing Electricity from Beverage". Society for Science and the Public. Retrieved 2015-12-25.
- ↑ http://www.cbc.ca/news/technology/ann-makosinski-s-new-invention-a-body-heat-powered-headlamp-1.2678576
- ↑ "Global Thermoelectric Generator Market is estimated to cross US $720 million by 2021: by Market Research Engine". www.keyc.com. Retrieved 2015-10-28.
- ↑ "Thermoelectric Generators Market Worth 547.7 Million USD by 2020". www.prnewswire.com. Retrieved 2015-10-28.
- ↑ "Sub-watt thermoelectric generator market on the up". 2016-03-15. Retrieved 2016-09-13.
External links
- Small Thermoelectric Generators by G. Jeffrey Snyder
- Kanellos, M. (2008, November 24). Tapping America’s Secret Power Source. Retrieved from Greentech Media, October 30, 2009. Web site: http://www.greentechmedia.com/articles/read/tapping-americas-secret-power-source-5259/
- LT Journal October 2010: Ultralow Voltage Energy Harvester Uses Thermoelectric Generator for Battery-Free Wireless Sensors
- Biolite Camp Stove - Biofuel stove and Thermogenerator in one.
- Description of Thermoelectric Generators
- Fire Powered TEGs - Hatsuden Nabe
- Wood Stove Thermoelectric Generators
- Thermoelectric Generator Energy Harvesters
- DIY: How to Build a Thermoelectric Energy Generator With a Cheap Peltier Unit